Mechanism and Application of High-Temperature Copper Diffusion in Surface Recoloring of Labradorites
Keywords: Copper diffusion, labradorites, copper nanoparticles
Feldspar, with the chemical formula of [KAlSi3 O8 ]x[NaAlSi3 O8 ]y[CaAl2 Si2 O8 ]z (x + y + z = 100), is one of the most important rock-forming minerals in the earth’s crust, and those of high quality can be used as gemstones. The main feldspar gems on the market include moonstone, sunstone, amazonite, and iridescent rainbow labradorite. Among them, sunstone exhibits a strong golden and red metallic luster due to microscopic metallic inclusions. But there is a special kind of labradorite sunstone ([NaAlSi3 O8 ]30–50[CaAl2 Si2 O8 ]50–70) that not only shows “schiller”, but also presents brilliant red and green bodycolors. Currently, the reported localities of this kind of natural labradorite sunstone are the U.S. state of Oregon and the Afar region of Ethiopia (Johnston et al., 1991; Kiefert et al., 2019; Sun et al., 2020). This material is highly valued and favored by many gem carvers and collectors for its unique optical properties. As for the color origin of labradorite sunstone, authors CW and AHS (Wang et al., 2019) from the present study directly observed the microscopic morphology of Cu nanoparticles (NPs) in natural sunstone by means of Focused Ion Beam – Transmission Electron Microscopy (FIB-TEM ).
It is also because of the high price and huge market potential of natural sunstones that an artificially diffused red andesine feldspar was unveiled during the 2006 Tucson gem shows (Rossman, 2011). The diffused red andesine feldspar has the same coloring mechanism (Cu NPs) as natural sunstone. But the treatment methods on the market are not completely understood. Many researchers have tried to generate red and green colors using copper diffusion experiments, typically by placing colorless or light-yellow feldspar in a zirconia powder containing a small amount of copper and then performing a high-temperature diffusion treatment (Emmett et al., 2009; Thirangoon, 2009). In this abstract, we demonstrated the mechanism and application of high-temperature copper diffusion in surface recoloring of labradorites.
The high-temperature diffusion process was divided into three stages according to the color change of labradorite (Figure 1b).
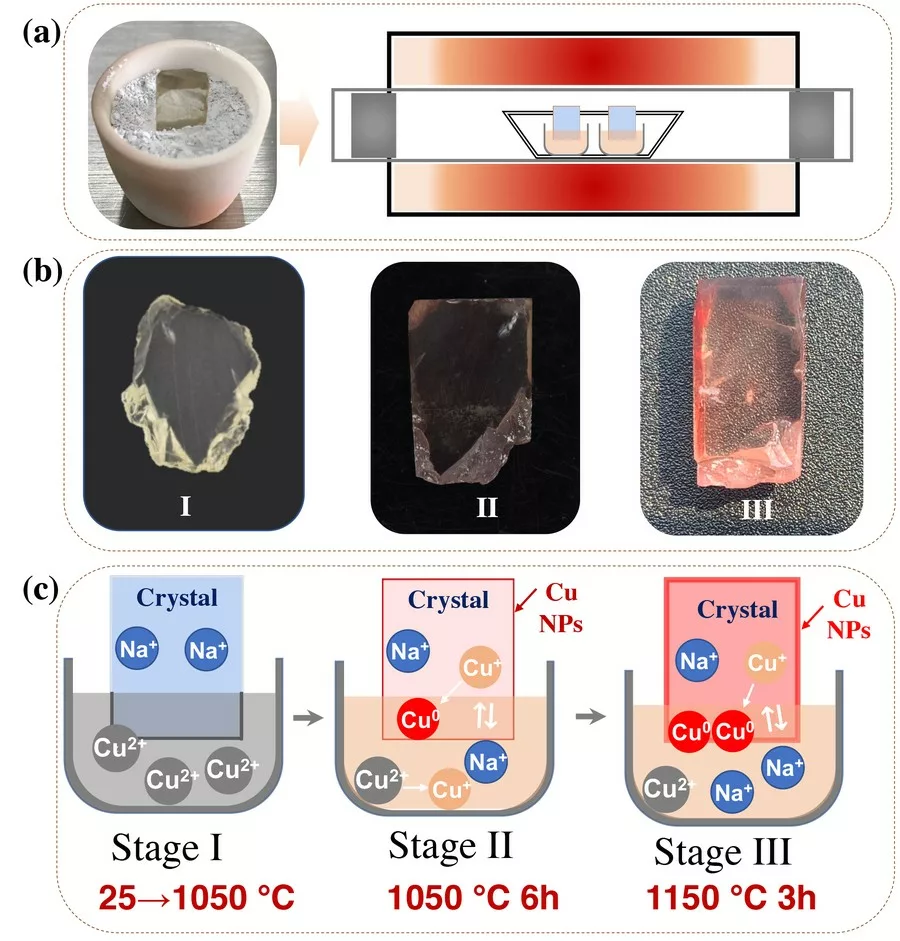
In stage I, the temperature of the tube furnace gradually increased from room temperature to 1050 °C. When the labradorite was taken out from the tube furnace as soon as the temperature reached 1050 °C, the color has not changed. According to experimental results and thermodynamic calculations, when the temperature is lower than 1050 °C in air atmosphere, Cu2+ ions with 3d9 electronic configuration is more stable (Rakhshani, 1986); when the temperature is higher than 1050 °C, Cu+ with 3d10 electronic configuration is more stable. Therefore, the chemical state of the CuO in stage I will remain unchanged within the temperature range of 25~1050 °C. Obviously, it is difficult for Cu2+ ions to diffuse into the labradorite.
In stage II, the temperature of the tube furnace is maintained at 1050 °C for 6 h, and the labradorite became light red when they were taken out from the tube furnace. Since the temperature is higher than 1050 °C, the transition of Cu2+→Cu+ could occur from the perspective of thermodynamics. Therefore, we inferred here that copper enters the labradorite in the form of Cu+ ions. The diffusion of Cu+ions can also be confirmed by the fluorescence spectra. The labradorite after the high-temperature diffusion process have fluorescence peaks at ~394 nm and ~555 nm. According to literature reports, the fluorescence emissions at ~394 nm and ~555 nm are caused by Cu+ and Cu+ -Cu+ dimer respectively (Lutz et al., 1997; Puppalwar et al., 2011). And the Cu+ ions entered in the labradorite could be further reduced to Cu0 by capturing hot electrons generated in the labradorite under high temperatures. After holding for 6 h at 1050 °C, with continuous entering and reduction of Cu+ ions, the amount of Cu0 aggregated on the surface of labradorite reached the critical point, which will lead to the formation of Cu NPs. The LA-ICP-MS data confirm that Cu+ ions diffuse into the labradorite through Cu+ -Na+ ions exchange.
In stage III, the temperature of the tube furnace is increased to 1150 °C, then maintained at this temperature for 3 h, and the labradorite became red when they were taken out from the tube furnace. In this stage, the effect of temperature on the diffusion rate is very important. An increase of 100°C is beneficial to the formation and growth of Cu NPs, since the temperature rise will accelerate the process of Cu+ ions entering the labradorite and being reduced to the form of Cu0 . The surface plasmon resonance (SPR) absorption peak of the labradorite obtained in stage III is obviously enhanced after holding for 3 h at 1150 °C. The TEM and EELS data revealed that spherical Cu NPs with an average size of 46±5.9 nm were finally produced in the labradorite. The surface of two faceted labradorites were recolored by using a similar high-temperature copper diffusion process. In order to have a more direct comparison with the sample before copper diffusion, the images of the faceted labradorites before and after the high-temperature copper diffusion are presented in Table 1.
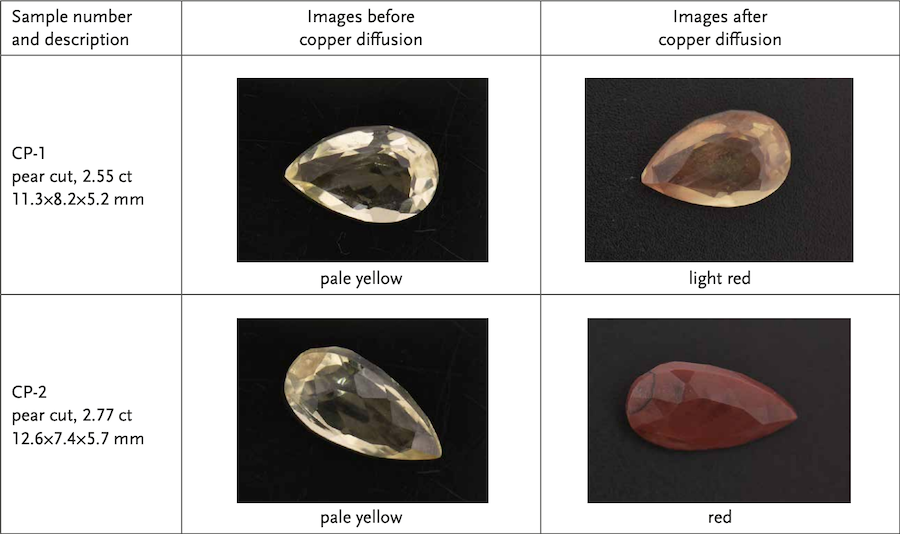
References:
- Emmett, J., Douthit, T., 2009. Copper diffusion in plagioclase. GIA News from Research. Aug. 21
- Johnston, C.L., Gunter, M.E., Knowles C.R., 1991. Sunstone labradorite from the Ponderosa mine, Oregon. Gems & Gemology, 27(4), 220–233.
- Kiefert, L., Wang, C., Sintayehu, T., Link, K., 2019. Sunstone labradorite-bytownite from Ethiopia. Journal of Gemmology, 36(8), 694–696.
- Lutz, T., Estournes, C., Merle, J. C., Guille, J. L., 1997. Optical Properties of Copper-Doped Silica Gels. Journal of Alloys and Compounds, 262, 438-442.
- Puppalwar, S. P., Dhoble, S. J., Kumar, A., 2011. Improvement of Photoluminescence of Cu+ Ion in Li2 SO4 . Luminescence, 26(6), 456-461.
- Rakhshani, A. E., 1986. Preparation, Characteristics and Photovoltaic Properties of Cuprous Oxide—A Review. SolidState Electronics, 29(1), 7-17.
- Rossman, G.R., 2011. The Chinese red feldspar controversy: Chronology of research through July 2009. Gems & Gemology, 47(1), 16–30.
- Sun, Z., Renfro. N.D., Palke. A.C., Breitzmann. H., Muyal. J., Hand. D., Hain. M., McClure. S.F., Katsurada. Y., Miura. M., Rossman. G.R., 2020. Gem News International: Sunstone plagioclase feldspar from Ethiopia. Gems & Gemology, 56(1), 184–188.
- Wang, C., Shen, A.H., Palke, A.C., Heaney, P.J., 2019. Color origin of the Oregon sunstone. In Proceedings of the 36th International Gemmological Conference IGC, Nantes, France, August 27–31, pp. 71–74.